Surface melt and ponding on Larsen C Ice Shelf and the impact of föhn winds | Antarctic Science | Cambridge Core
Abstract
A common precursor to ice shelf disintegration, most notably that of Larsen B Ice Shelf, is unusually intense or prolonged surface melt and the presence of surface standing water. However, there has been little research into detailed patterns of melt on ice shelves or the nature of summer melt ponds. We investigated surface melt on Larsen C Ice Shelf at high resolution using Envisat advanced synthetic aperture radar (ASAR) data and explored melt ponds in a range of satellite images. The improved spatial resolution of SAR over alternative approaches revealed anomalously long melt duration in western inlets. Meteorological modelling explained this pattern by föhn winds which were common in this region. Melt ponds are difficult to detect using optical imagery because cloud-free conditions are rare in this region and ponds quickly freeze over, but can be monitored using SAR in all weather conditions. Melt ponds up to tens of kilometres in length were common in Cabinet Inlet, where melt duration was most prolonged. The pattern of melt explains the previously observed distribution of ice shelf densification, which in parts had reached levels that preceded the collapse of Larsen B Ice Shelf, suggesting a potential role for föhn winds in promoting unstable conditions on ice shelves.
...
Discussion
The pattern of increasing surface melt duration, earlier melt onset, and later freeze onset from East–West on Larsen C Ice Shelf, and the high concentration of melt in the western ice shelf inlets may be explained by the föhnwarming effect resulting from predominantly westerly winds (Munneke et al. 2012, Barrand et al. 2013). A shift towards increasingly positive phase of SAM over the past c. 50 years has induced stronger circumpolar westerlies, enhancing the frequency and/or amplitude of föhnwarming events to the east of the Antarctic Peninsula (Orr et al. 2004, Marshall et al. 2006, Turner et al. 2013). The overall effect of föhn winds is to enhance leesidesurface melt via enhanced surface solar radiation and sensible heat fluxes. The greatest föhn-induced melt should be expected to be close to the base of the Peninsula’s lee slopes due to greater near-surface air temperatures, and beneath föhn jets due to stronger near-surface winds and more frequent occurrences of föhn reaching the surface here. Note this is despite the jets being slightly cooler than adjacent calmer regions of föhn air; a result of lower, potentially cooler source altitudes for the föhn jets, which are the downwind continuation of gap winds across lower sections or ‘gaps’ in the Antarctic Peninsula’s crest (Elvidge et al. 2014). The distribution of leeside warming and winds evident in the case studied here qualitatively resembles that of another westerly föhnevent over the Antarctic Peninsula a week earlier on 27–28 January 2011 (Elvidge et al. 2014). A stronger case of cross-Peninsula westerlies exhibits some significant differences in dynamics and leeside response, though the key elements of greatest temperatures at the foot of the lee
slopes and strongest winds within the inlets are consistent (Elvidge et al. 2014). The resulting gradient in surface melt explains, in turn, the pattern of ice shelf densification observed by Holland et al. (2011) who showed a corresponding lower air content towards the north of Larsen C Ice Shelf and particularly in the north-western embayments including Cabinet Inlet. This part of the ice shelf is approaching the densification observed in Larsen B Ice Shelf immediately prior to its collapse (Holland et al. 2011), suggesting a possible link between föhn-enhanced surface melt and potentially unstable ice shelf conditions. Our observations of melt ponds are also spatially consistent with this focus in melt duration. Where surface melt is most concentrated the ice shelf has become most dense, and is most able to both generate and sustain melt ponds on the surface. There are two possible hypotheses to explain the rapid disappearance of the melt ponds. The first is that they freeze over at the end of summer and rapidly become covered by snow which obscures them from optical satellite instruments. The second is that, in a similar way to melt lakes on the Greenland ice sheet, they drain through fractures in the ice shelf, possibly as a result of hydrofracture (e.g. Das et al. 2008). The ice in this part of the shelf has near-zero air content (Holland et al. 2011) and its dynamic regime is compressive (Jansen et al. 2010). This favours the first hypothesis because the melt
ponds are observed in the SAR data at the end of summer. However, the alternative hypothesis cannot be completely ruled out. If near-zero air content is a pre-condition for ice shelf disintegration then the coincident melt ponds are already commonly present to provide a possible trigger for collapse (MacAyeal & Sergienko 2013), and there may already be fractures through the full ice thickness in response to hydrofracture. Future monitoring of ice shelf melt ponds is therefore highly desirable. The investigations of Larsen C Ice Shelf show that melt ponds are observable in optical satellite data but the predominant cloud conditions and the potentially short window of opportunity to observe these ephemeral features means that an alternative method of monitoring them is required. Data from SAR of sufficient spatial resolution and temporal sampling frequency may be usedto perform this role. The launch of ESA’s Sentinel 1 mission in 2014 may sufficiently extend the archive of suitable data to allow trends in melt pond generation to be explored in future. Melt ponds are observed up to tens of kilometres in length and are aligned with the flow direction suggesting that they congregate in surface depressions arising from ice flow and/or basal melt processes. Their size suggests that a reservoir of water can regularly form which may be sufficient to sustain the hydrofracture process, although further research is required on the small-scale topography of the inlets to
properly characterize melt pond depth and volume. In conclusion, using a novel source of satellite microwave imagery and meteorological modelling we have highlighted, and provided an explanation for, the concentration of surface melt and ponding on the western inlets of Larsen C Ice Shelf. This pattern of melt helps to account for the distribution of densification observed by Holland et al. (2011) and highlights a potential contributing process to the collapse of ice shelves elsewhere.
Related Content
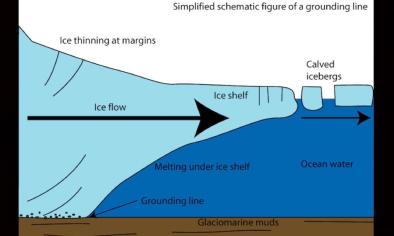
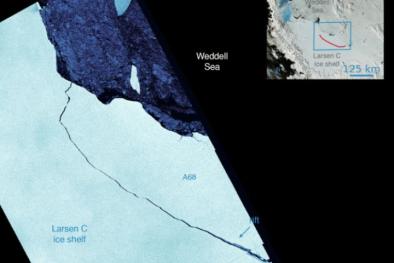
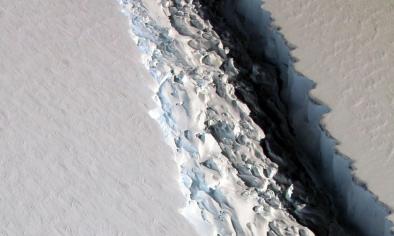
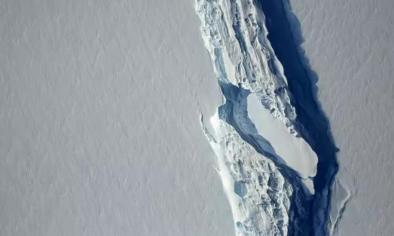